All published articles of this journal are available on ScienceDirect.
Altering Amino Acid Profile in Catharanthus Roseus (L.) G. Don Using Potassium and Ascorbic Acid Treatments
Abstract
Background:
Catharanthus roseus (L.) G. Don is the main source of alkaloids anticancer drugs. Alkaloids are derived from amino acids and can lead to changes in these valuable compounds.
Objective:
This experiment evaluated the variation of amino acids under potassium and ascorbic acid treatments.
Methods:
Different concentrations (1.5, 3.16, 15 and 30 mM) and forms (K2SO4 and KNO3) of potassium (K+) were added to plants via Hoagland’s nutrient solution. Ascorbic acid (AsA) (750 mg L-1) was sprayed on the leaves surfaces on days 68 and 78. Amino acids were extracted from 90-day-old plant leaves, and different amino acids were determined by High-Performance Liquid Chromatography with fluorescence detection.
Results:
Amino acids increased in K+ deficiency (1.5 mM), but changes in negatively charged amino acids were lower. In contrast, N-rich amino acids showed the biggest change. In excessive K+, branched-chain and aromatic amino acids decreased, while the least amount of the other amino acids was observed in the plants treated with optimum K+.
Conclusion:
The exterior of AsA and excessive K+ cause branched-chain and aromatic amino acids, aspartic acid and glutamic acid to decrease.
1. INTRODUCTION
Catharanthus roseus (L.) G. Don (C. roseus) belongs to the family of Apocynaceae. It is an important medicinal plant and the main source of several alkaloid anticancer drugs [1]. The alkaloids are derived from amino acids [2], so changes in the number of amino acids can lead to changes in these valuable compounds. The importance of the pool size of free amino acids is related to the role of free amino acids in protein biosynthesis as well as their functions in metabolic pathways [3]. Some amino acids act as precursors of the biosynthesis of nitrogenous compounds, such as secondary metabolites [4]. Evidences showed that abiotic stress, heavy metals and macronutrients can lead to changes in the amount of free amino acids in plants. It was reported that alanine and glutamate highly accumulated during water logging in Lotus japonicus [5]. Arabidopsis plants treated with cadmium displayed increased levels of alanine and serine as well as other metabolites [6]. It was reported that under chromium treatment, ornithine and alanine accumulated in rice [7]. In addition to heavy metals, macronutrients can also change the number of free amino acids [8]. It is a well-known fact that the mineral nutrition of plants affects amino acid content and protein in plants. The deficiency of phosphorus and potassium generally led to an increase in the number of amino acids compared to the control plants [9]. For example, in common bean, more amino acids increased under phosphor deficiency [10].
Potassium (K+) is one of the most macronutrients that alter physiological and biochemical processes in plants [11]. K+ affects the number of proteins and free amino acids [8]. The amount of protein decreases in plants with K+ deficiency [12], so the amount of free amino acids is higher in K+-sufficient plants [13]. Several amino acids, such as leucine, isoleucine, valine, arginine, threonine and lysine, accumulated during K+ deficiency in Arabidopsis [14], while the amount of glutamic acid and aspartic acid is lower than other amino acids [15]. K+ increases lysine content and decreases the amount of methionine in the wheat plant [16]. K+ is not only a constituent of the plant structure, but also has a regulatory function in several biochemical processes related to protein synthesis [17]. Protein-synthesizing enzymes have specific needs for K+ because K+ corrects the structure of enzymes to have better efficiencies in related pathways [18].
Ascorbic acid (AsA) also affects the primary and secondary metabolites [19], such as alkaloids [20], proteins and amino acids [21]. AsA has an important role in stabilizing the structure of cell-like proteins [22]. The role of exogenous AsA in spinach (Spinacia oleracea L.) showed that AsA causes phenylalanine to decrease. AsA-treated plants can enhance the secondary metabolism; this change is supported by higher consumption of phenylalanine [23]. AsA has a key role in different stresses, such as oxidative [24], hyperosmotic [25] and anoxic [5] stresses. So, AsA can affect response to these stresses. For example, under hyperosmotic conditions, the transcription of the genes encoding enzymes are involved in arginine biosynthesis [25] and asparagine synthase in sunflower increased [26], while the others that are involved in the degradation of asparagine decreased.
Although the role of K+ and AsA in amino acid metabolism in different plants has been reported [8], there is not any report on their effects in these components in C. roseus. Since different amino acids contributed to the production of secondary metabolites, the concentration of these metabolites is important for the production of anticancer alkaloids in C. roseus. This study was conducted to evaluate the effect of K+ and/or AsA on different groups of amino acids such as acidic or negatively charged (aspartic acid and glutamic acid), N-rich or positively charged (arginine and ornithine), aromatic (tryptophan and phenylalanine) and branched-chain (valine, leucine, isoleucine) amino acids in C. roseus.
2. MATERIALS AND METHODS
2.1. Plant Materials and Treatments
C. roseus seeds are germinated in Petri dishes using a water agar culture medium. Uniform 3-day-old germinated seeds were transferred to pots filled with perlite and irrigated with half-strength Hoagland’s nutrient solution. The seedlings were kept in a growth chamber at 28 ºC and 65% relative humidity for (light/dark) photoperiod 16/8 h at an irradiance of 450 µmol m−2 s−1. Different potassium concentrations as treatment were added to Hoagland’s nutrient solution in the range of 1.5 (deficient), 3.16 (optimum), 15 and 30 mM (excessive) of K+ in the forms of KNO3 or K2SO4. Ascorbic acid (AsA) was manually sprayed twice to the surface of the leaves. The first time when the plants were 68 days old, and the second time when they were 78 days old. The concentration of AsA in the solution was 750 mg L-1 (containing 0.1% Tween 20 as a surfactant agent). Spraying took place until dripping from the leaves. The plant samples were collected at harvest time when the plants were 90 days old.
2.2. Sample Preparation for Analysis of Plant Free Amino Acids by HPLC
2 g C. roseus fresh frozen leaves were homogenized with 10 mL methanol containing 2% formaldehyde using an ultra-homogenizer (Heidolph, Silent Crusher M, Germany) at 10000 rpm in an ice bath for 5 min. After incubation for 2 h, the samples were centrifuged at 13000 rpm for 10 min. The supernatants were separated and dried completely using a rotary evaporator at 40 ºC under reduced pressure (40 mbar). Next, 1 mL 14% ammonia was added to unionize the alkaloids, raising their hydrophobicity. Amino acids were back-extracted seven times with 1 mL diethyl ether. After that, the residual aqueous part on Na2SO4 dried completely and was solubilized in HPLC Mobile Phase A and B (1:1) by1 mL prior to HPLC analysis [27].
2.3. Determination of Free Amino Acids Profile
The plant leaves extract was used to determine the amino acid profile (Figs. 1 and 2). HPLC unit: solvent delivery was pump 6.1L, Azura Knauer (Germany). Shimadzu Prominence RF-20A was used as a fluorescence detector (Japan) (emission 450 and excitation 430 nm wavelengths), CTO-20A, Prominence, Shimadzu Corp. (Japan) as column oven. Rheodyne 7725i, Perkin Elmer (USA), fitted sample-loading loop 50 µL, C18 reversed-phase column (150 × 4.6 mm), Nucleodur C18, Gravity 5 µm, Macherey-Nagel (Germany), and clarity software 5 as LC workstation, SGE 50 µL as Syringe (Australia) were used. The method was pre-column OPA derivatization. The internal standard was norleucine. The sample injection volume was 50 µL at a flow rate of 1.2 mL min−1. Three plant samples were used for each determination. Mobile phase A: 1.02 g potassium dihydrogen phosphate and 0.43 g dipotassium hydrogen phosphate in 500 ml ultrapure water. Mobile phase B: 50 mL ultrapure water, 250 mL acetonitrile, and 200 mL methanol [28].
2.4. Statistical Analysis
The experiments were performed as a factorial layout in a completely random design with 3 replicates. Statistical analysis was performed using SPSS 22. Duncan’s multiple test ranges were used for mean comparison and Excel 2016 was used to draw the necessary figures.
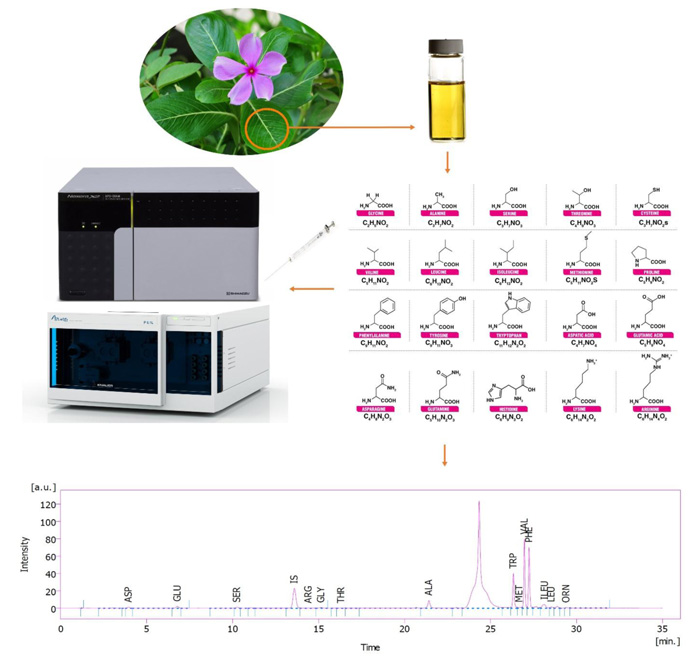
3. RESULTS
3.1. Effect of K+ and AsA on Branched-Chain and Aromatic Amino Acids
Branched-chain and aromatic amino acids, as well as alanine, exhibited a similar pattern in K+ treated plants. The amount of these amino acids correlated inversely with the amount of K+. In the K+ deficient-plant, the amount of valine, leucine, isoleucine, tryptophan, phenylalanine and alanine was higher than that of the plants with optimum K+ (3.16 mM K+), while excessive K+ (15 or 30 mM) caused these amino acids to decrease significantly (Figs. 3 and 4). AsA had a significant effect (P ≤ 0.05) on the amount of branched-chain and aromatic amino acids and alanine (Table 1). Using AsA with any concentration of K+ (in deficient, optimum or excessive K+) reduced the amount of these amino acids compared to the plants with no AsA (Table 1). It was observed that the dual effect of K+ and AsA had an interaction with and a significantly reduced effect (P ≤ 0.05) on the amount of valine, leucine, isoleucine, tryptophan, phenylalanine and alanine compared to the optimum condition (no AsA and optimum K+) (Fig. 4).
3.2. Effect of K+ and AsA on Negatively Charged and N-rich Amino Acids
There were significant differences (P ≤ 0.05) in the amount of negatively charged, positively charged (N-rich) and some other amino acids (Figs. 3 and 4) due to the concentration of K+. Although negatively charged amino acids showed the least change, the most significant change was related to the N-rich amino acids as well as serine, threonine, glycine and methionine. In K+ deficiency, aspartic acid and glutamic acid increased less than other amino acids; however, more increase was related to serine, arginine, threonine, glycine, methionine and ornithine compared to the control plants. Similar to K+ deficiency, in excessive K+ (15 or 30 mM) increase in aspartic acid and glutamic acid was very low, while serine, arginine, threonine, ornithine and glycine showed more increase compared to the control plants (Figs. 3 and 4). Spraying AsA resulted in decreasing the amount of negatively charged amino acids compared to the optimum plants (3.16 mM K+ with no AsA). The amount of more amino acids in treated plants with AsA in all concentrations of K+ was less than the plants with no AsA (Fig. 4). However, the result indicated that most amino acids showed a higher reduction in treated plants with AsA in excessive K+ than in deficient K+ (Table 1). The dual effect of K+ and AsA had a significant interaction (P ≤ 0.05) with the amount of the mentioned amino acids. The amount of glycine, serine, and ornithine increased significantly; however, aspartic acid and glutamic acid increased less than the others compared to the optimum condition (Fig. 4).
Amino acids | K+ deficiency |
Optimum condition (3.16 mM K+) |
Excessive K+ |
---|---|---|---|
Leucine | 24 | 12 | 57 |
Isoleucine | 28 | 19 | 31 |
Alanine | 48 | 20 | 55 |
Phenylalanine | 26 | 25 | 40 |
Valine | 38 | 35 | 69 |
Tryptophan | 35 | 36 | 53 |
Serine | 12 | 0.6 | 34 |
Glutamic acid | 0.02 | * | 24 |
Aspartic acid | 0* | * | 27 |
Ornithine | 44 | * | 60 |
Arginine | 36 | * | * |
Threonine | 28 | * | * |
Glycine | 17 | * | 26 |
Methionine | 20 | * | * |
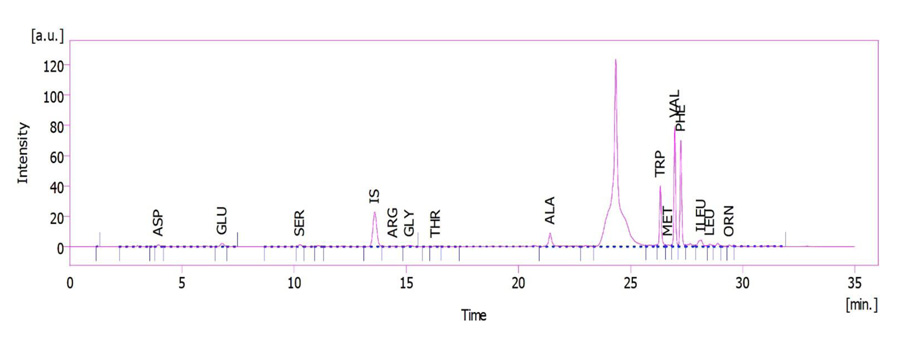
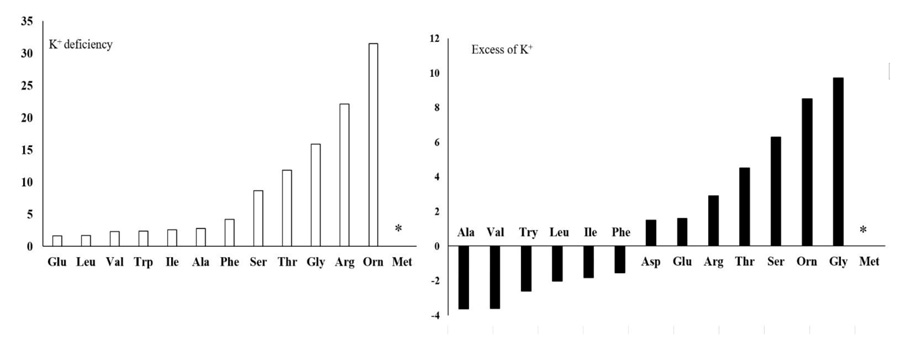
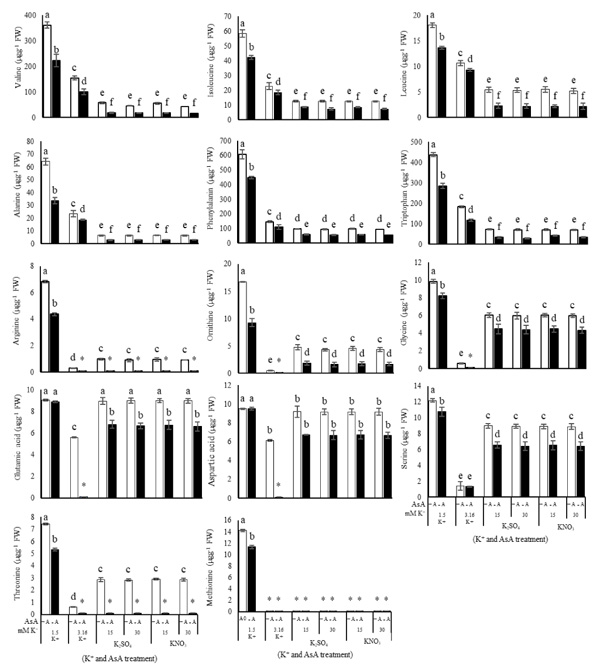
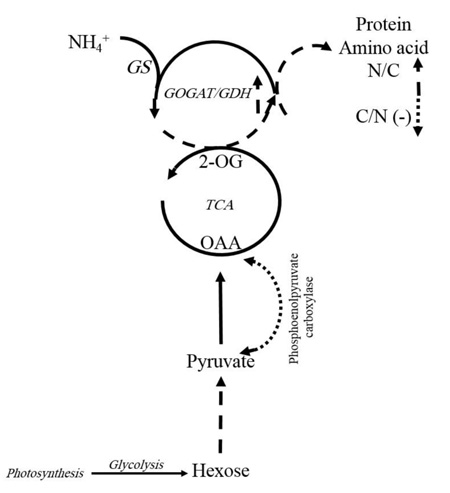
4. DISCUSSION
Although free amino acids are very important due to their need for protein biosynthesis and their contribution to different metabolic pathways, they may change in response to environmental factors. For example, free amino acids accumulated sharply in K+ deficiency because protein degradation accelerates to form free amino acids, and amino acids could not be used successfully to synthesize proteins in K+ deficiency. There is an increase in the accumulation of free amino acids in more plants in response to stresses. In this experiment, not only K+ deficiency increased the amino acids, but different concentrations of K+ had different effects on different amino acids content.
Generally, free amino acids such as branched-chain (valine, leucine, and isoleucine), aromatic (tryptophan and phenylalanine), positively charged or N-rich (arginine and ornithine) amino acids, as well as alanine, glycine, threonine and methionine significantly increased (P ≤ 0.05) under K+ deficiency (1.5 mM K+). K+-deficient plants usually have a higher content of soluble nitrogen compounds, free amino acids and amides compared to those supplied with adequate K+ [13]. In cotton leaves, free amino acid content increased, but soluble protein significantly decreased with K+ deficiency. We observed that in K+ deficiency, negatively charged amino acids such as glutamic acid and aspartic acid increased less than the other amino acids, especially the positively charged (N-rich) amino acids like arginine and ornithine. This result is similar to the report for Arabidopsis, which showed more amino acids except that glutamic acid and aspartic acid increased under K+ deficiency [14]. In contrast, there was an increase in positively charged amino acids like arginine in wheat, which is probably related to its protective role during stress [24]. A reason for less accumulation of negatively charged amino acids such as aspartic acid and glutamic acid, in contrast to N-rich amino acids in K+ deficiency, might be explained as follows: It was observed that in K+ deficiency, the amount of free amino acids increased, but the amount of nitrate and ammonium in leaf significantly decreased [29]. When C3 plants need to produce organic acid for amino acid biosynthesis, phosphoenolpyruvate carboxylase plays an important role in the special process that results in the conversion of phosphoenolpyruvate to oxaloacetate. So, under K+ deficiency, the biosynthesis of malate and citrate is limited by lower phosphoenolpyruvate carboxylase activity. This function of K+ can be due to the contribution of K+ as a cofactor in some enzyme activities, adjustment of the balance between cations and anions, and the correction of enzymes structure to have better efficiency [18], which leads to a decrease in C-flux into the amino acids [29]. On the other hand, as 2-oxoglutarate contributes to the amino acid process, so under K+ deficiency, reduction in malate and citrate can lead to a reduction in 2-oxoglutarate. As a result, C/N ration limits amino acid synthesis in leaves. This could be a reason for less accumulation of negatively charged amino acids such as aspartic acid and glutamic acid in contrast to N-rich amino acids in K+ deficiency. Protein content significantly decreased with K+ deficiency [30]. One possibility is that changes in metabolites are an active stress response to reprogramming of metabolism in plants. This indicated that the regulation of some enzymes present in catabolism and anabolism of amino acids are affected in the concentrations of metabolites under stressful conditions [14]. Several dynamic programmings of metabolism occur downstream of glycolysis, TCA, and GS/GOGAT/GDH cycles that nearly express the reason for increasing total amino acids (Fig. 5). Enzymes preserve carbon flux into amino acids and proteins, decrease negatively charged amino acids and increase enriched amino acids. Therefore, the regulation of enzymes is so crucial in the plant’s adaptation to K+ deficiency [14]. Any change in related pathways can lead to an increase in N-rich amino acids such as arginine and ornithine compared to negatively charged amino acids like glutamic acid and aspartic acid, which was observed in our results.
Excessive K+ (15 or 30 mM) resulted in decreasing branched-chain amino acids such as isoleucine, leucine and valine, as well as aromatic amino acids like tryptophan and phenylalanine, while aspartic acid, glutamic acid, serine, arginine, threonine, ornithine and glycine increased compared to the control plants. Although the amount of these amino acids increased in excessive K+ compared to optimum conditions, their amounts are much lower than in the K+ deficient plants (Figs. 3 and 4). It was reported that in the K+ deficient tobacco, soluble amino acids are accumulated [14]. Although stressful conditions such as K+ deficiency, increasing all amino acids, it seems that in sufficient K+ the best concentration for synthesis or the prevention of degradation of each amino acid is different from other amino acids. In this regard, it was reported that K+ possesses a positive impact on lysine content but no impact on methionine in wheat [16]. So, it can be concluded that the best conditions for overcoming synthesis and decreasing degradation of any amino acid or protein are different from each other. It can be a reason for different patterns of branched-chain and aromatic amino acids in excessive K+. We observed that K+ deficiency and excessive K+ had the least change in the amount of branched-chain amino acids. Branched-chain amino acids such as valine, leucine and isoleucine are synthesized by plants in a protected process, and this process has a feedback inhibition. So, it can be concluded that any change in the amount of branched-chain amino acids in different conditions cannot be highly variable.
Spraying AsA under optimum K+ (3.16 mM K+) decreased the amount of branched-chain and aromatic amino acids as well as alanine, glutamic acid and aspartic acid compared to the plants with no AsA. Branched-chain amino acids are precursors of the biosynthesis of secondary metabolites in various plants, and there are many secondary metabolites (alkaloids) in C. roseus. So, decreasing aromatic and branched-chain amino acids can be the result of further production of secondary metabolites in AsA-treated plants.
As primary metabolites, amino acids contribute to the modification of the harmful situations under several stress conditions. For example, the accumulation of amino acids as osmolytes improves the status of water potential in plants [24]. Under hyperosmotic conditions, the transcription of the genes encoding the enzymes that are involved in arginine biosynthesis [24] and asparagine synthase in sunflower [26] increased, while those involved in the degradation of asparagine decreased. Increasing the level of nitrated amino acids is an early sign of lesion that occurs related to oxidative stress in plants, even in the presence of endogenous antioxidants [30]. Free radicals which are produced under stressful conditions result in the oxidation of proteins. AsA is one of the most important antioxidant substances in plants, so it can significantly decrease oxidizing radicals [24]. As a result, it can effectively decrease amino acids since amino acids are precursors of proteins. So, an increase in the amount of proteins could be associated with a decrease in all amino acids compared to the plants treated with K+ and not with AsA.
In the dual effect of K+ and AsA, the amount of branched-chain and aromatic amino acids decreased, but the amount of aspartic acid, glutamic acid, glycine, serine and ornithine increased when compared to the control plants (3.16 mM K+ with no AsA). Different groups of proteases break proteins into amino acids [3]. K+ acts as a cofactor in some enzymes in metabolism pathways and adjusts the balance between cations and anions in some processes. As a result, the optimum concentration of K+ creates an optimum condition for enzymes in metabolic pathways to have a better condition in the plant metabolism. Simultaneously, AsA acts as a cofactor for some enzymes, such as enzymes involved in protein biosynthesis [19]. It seems that the exact and final levels of amino acids are a key point for the regulation of proteins and synthesis and catabolism of amino acids. In this regard, the amount of carbon and nitrogen and the defense system as well as development of plants are dependent on the regulation of fluxes, transport and content of amino acids in plants.
CONCLUSION
As a consequence, it seems that K+ and AsA create a better condition for plants with two favors: a) it prevents degradation of proteins, b) it produces more products from free amino acids. Amino acids have different roles in plants. Some of them are signaling molecules, while others act as precursors in secondary metabolites biosynthesis. Regarding the fact that some amino acids like tryptophan contribute to alkaloids biosynthesis, such as vinblastine and vincristine in C. roseus, any change in amino acid content in this plant can be very valuable.
ETHICS APPROVAL AND CONSENT TO PARTICIPATE
Not applicable.
HUMAN AND ANIMAL RIGHTS
Not applicable.
CONSENT FOR PUBLICATION
Not applicable.
AVAILABILITY OF DATA AND MATERIALS
All data generated or analyzed during the present study are included within the article.
FUNDING
None.
CONFLICT OF INTEREST
The authors declare no conflict of interests, financial or otherwise.
ACKNOWLEDGEMENTS
Thanks to Dr. Hossein Salmanizadeh, Mahdieh Medical Diagnostic Centre, Isfahan, Iran for consulting HPLC analysis and methods, and the Biology Department of the University of Isfahan for lab facilities and financial support.