Pyridoxine Decreases Oxidative Stress on Human Erythrocyte Membrane Protein in vitro
Abstract
Background:
Pyridoxine has reduction and prevention against the levels of reactive oxygen species in in vitro studies. However, the biochemical mechanism that explains this behavior has not yet been fully clarified.
Objective:
To evaluate the effect of pyridoxine against oxidative damage on the membrane of human erythrocytes.
Methods:
Cumene hydroperoxide was used to induce oxidative stress in protein and lipid. Human erythrocytes were incubated with pyridoxine and cumene hydroperoxide, either alone or together for 8 h. Oxidative damage was determined by measuring lipid peroxidation and membrane protein carbonylation.
Results:
The results indicate that the malondialdehyde concentration decreased with increasing concentration of pyridoxine. The membrane protein content also decreased with increasing concentration of vitamin B6, which was confirmed by the decreased signal intensity in the western blot when compared to control without pyridoxine. Results demonstrate that pyridoxine can significantly decrease lipid peroxidation and protein carbonylation in red cell membrane exposed to high concentrations of oxidant agent.
Conclusion:
Pyridoxine showed a protective effect against the oxidative stress in human erythrocytes in vitro, inhibiting the carbonylation and the oxidative damage of erythrocyte membrane proteins. To date, such an effect has not yet been reported in terms of protein oxidation.
1. INTRODUCTION
The accumulation of free radicals in the oxidative stress plays a crucial role in the development of many diseases like cancer, arteriosclerosis, arthritis, neurodegenerative disorders, diabetes, hypertension and other conditions [1]. Erythrocyte is used in most of these conditions to investigate oxidative damage due to its high vulnerability to peroxidation [2]. To protect against the damage caused by the presence of free oxygen radicals, erythrocytes have a very active antioxidant defense system consisting of enzymes and non-enzymatic compounds [1].
Another antioxidant defense system is the non-enzymatic system, which is classified into two classes: lipophilic (vitamin E, carotenoids, ubiquinone, melatonin, etc.) and water-soluble (vitamin C, glutathione, uric acid, ceruloplasmin, transferrin, haptoglobin, etc.). Three antioxidant vitamins A, C, and E provide defense against oxidative damage [1] and clinical trials have shown that administration of vitamins with antioxidant properties proved useful in protecting against oxidative stress in vivo [3, 4].
In the case of pyridoxine (vitamin B6), although it is not considered within the group of antioxidant vitamins, various studies have yielded promising results in its protective role against oxidative stress [5-12]. In clinical trials, vitamin supplements have proven beneficial in the symptoms of some chronic diseases [13-21]. However, although the role of pyridoxine in reducing the complications associated with diabetes, aging and neurodegenerative diseases, etc., has already been widely reported [13, 22, 23], the biochemical and molecular mechanisms underlying this process are poorly understood.
Recently, more evidence was provided on the antioxidant role of pyridoxine and its inhibition of reactive oxygen species [24-26]. Vitamin supplementation induces the transport of selenium and the activation of glutathione peroxidase in erythrocytes and muscles [27] and acts as a cofactor in the synthesis of cysteine, explaining its involvement in maintaining glutathione levels [28].
It has also been observed that pyridoxine is a strong inhibitor of singlet oxygen in the fungus Cercospora nicotiana [11] with comparable or superior effect on vitamins C and E, two of the most efficient known biological antioxidants [29]. in vitro, pyridoxine demonstrated reduced levels of reactive oxygen species, shown to be particularly inhibitory to superoxide radicals type, in high glucose concentrations in erythrocytes and in cultured lens cells [5, 30]. Moreover, it may also decrease lipid peroxidation caused by hydrogen peroxide in U937 monocyte cultures [10]. Some studies have supported its activity on the detoxification of heavy metals such as lead [31, 32] and others have demonstrated in vitro and in vivo, that pyridoxine lowers oxidative stress induced by chromium [6, 9]. In some organisms such as E. coli, C. nicotianae, S. cerevisiae, and A. thaliana, the mutation of genes involved in pathways of de novo synthesis and salvage of vitamin B6 showed increased sensitivity to the reactive species oxygen [33].
In recent studies, it has also been shown that pyridoxine is capable of reducing superoxide levels induced by H2O2 in cultured endothelial cells [12] and can also restore the migratory ability and stop oxidative stress in cultured endothelial cells under conditions of high levels of glucose [34].
Currently, more studies are needed to support the antioxidant role of pyridoxine in the red blood cell oxidative processes in human cells. Therefore, in this study, we evaluated the effect of pyridoxine in vitro on the antioxidant system in human erythrocytes and directed to the carbonylation of erythrocyte membrane proteins, which is an understudied mechanism. The results show that treatment with pyridoxine, significantly lowers the levels of methemoglobin, lipid peroxidation and protein carbonylation in erythrocytes treated with an oxidizing agent.
2. MATERIALS AND METHODS
2.1. Incubation of Erythrocytes
Erythrocytes were incubated at 5% hematocrit in PBS (Phosphate-buffered saline) supplemented with gentamicin and different final concentrations of the compounds studied (range = 100 µM - 0.1 µM) in a microplate, at 37° C, 5% CO2 and 90% relative humidity.
To determine the effect of pyridoxine on oxidative damage caused by cumene hydroperoxide (oxidizing agent), erythrocytes at 5% hematocrit were incubated for 1 hour with only pyridoxine (final concentration of 0.1 to 100 µM) and then after this time, were incubated for 10 hours with a constant concentration of 100 µM cumene hydroperoxide for all samples (initially 1 h with only pyridoxine and then 10 hours with pyridoxine and simultaneously cumene hydroperoxide). A sample of erythrocytes treated only with cumene hydroperoxide was used as a positive control and used as negative control, erythrocytes without any treatment.
2.2. Percentage of Hemolysis
Hemolysis was determined by measuring the absorbance of the hemoglobin released in the supernatants of the samples at a wavelength of 540 nm. For these tests, erythrocytes at 5% hematocrit were incubated independently for 2, 10 and 24 hours with pyridoxine and cumene hydroperoxide in a concentration range of 0.1 to 1000 μM, in order to find the ideal working range. A control solution with 5% distilled water hematocrit (100% relative hemolysis) was prepared, as previously described [35].
2.3. Methaemoglobin Content (metHb)
Oxidized hemoglobin (metHb) levels in erythrocytes were estimated using the measurement of haemolysate absorbance at 630 nm and at 700 nm and compared to the absorbance of haemolyzed erythrocytes treated with potassium ferricyanide at 58 μM (positive control) using 96-well microplates, as previously described [36].
2.4. TBARS Test
The TBARS assay is based on the determination of the Malondialdehyde concentration (MDA) in the samples [37]. MDA is a natural product of lipid peroxidation and is used as an indicator of oxidative stress. For this determination, the TBARS Assay Kit (Cayman) was used following the manufacturer's instructions.
2.5. Levels of Carbonylation of Proteins
The obtained erythrocytes were subjected to cell lysis, using 0.1% saponin in cold 1X PBS, followed by centrifugation at 10,000 x g for 15 min at 4°C. The supernatant was discarded and the resulting pellet (crude erythrocyte membranes) was washed 5 times with cold 1X PBS, centrifuging at 10000 x g for 10 min at 4°C. The supernatant was discarded and the membranes in the bottom were solubilized with 1X PBS. The determination of the carbonyl group content was based on the reaction between 2.4 dinitrophenylhydrazine (DNPH) and the carbonylated proteins [38]. For this determination, the Protein Carbonyl Colorimetric Assay Kit (Cayman) was used following the manufacturer's instructions.
2.6. Extraction and Preparation of Erythrocyte Membrane Proteins for Immunodetection of Carbonyl Groups
Identification of oxidative modifications of proteins by free radicals of oxygen and other reactive species was performed by Western Blot. Membrane proteins used to identify carbonylation levels of proteins from each sample were obtained by lysing the erythrocytes in 0.1% saponin in cold 1X PBS, followed by washing with cold 1X PBS and subsequent centrifugation at 10000 xg for 10 min at 4°C [39]. This step was repeated 4 times. The supernatant was discarded by aspiration and the pellet was resuspended in 100 μL of solubilization buffer (Ripa Lysis Buffer, Amresco®) supplemented with dithiothreitol and protease inhibitor and stored in aliquots at -20° C until used to make Western Blot.
2.7. Western Blot
Erythrocyte membrane proteins were derivatized with DNPH immediately after being obtained and stored in aliquots at −80°C until used. Carbonylated proteins were detected using antiDNPH antibodies by one-dimensional Western blotting analysis (OxyBlots), as previously described [39]. The non-derivatized proteins and the derivatized proteins were separated by 12% SDS-PAGE on independent gels. The gel of the non-derivatized proteins was stained using a Coomassie blue solution.
2.8. Statistical Analysis
Statistical analysis was performed using Sigma Plot 11.0 software. Results were expressed as means ± SD (standard deviation). For comparisons between two groups, Student's t-test was performed for parametric data and Mann-Whitney U test for non-parametric data. The Kruskal-Wallis test was used to compare more than two groups for non-parametric data, Values of p <0.05 were considered statistically significant.
3. RESULTS
3.1. Pyridoxine is not Toxic for Erythrocytes
The percentage of hemoglobin release (hemolysis) for the compounds used was less than 3% at concentrations below 100 μM (Table 1). Cumene hydroperoxide is toxic to erythrocytes and in these experiments, it caused cell lysis at a concentration of 1000 μM. Pyridoxine at the tested concentrations did not cause erythrocyte lysis. The changes compared to the untreated control were not statistically significant (p> 0.05, Student's t-test), for both pyridoxine and cumene hydroxide at concentrations less than 100 μM. In this way, concentrations of pyridoxine of 1, 10 and 100 μM were selected to evaluate the antioxidant effect to avoid the effect of hemolysis and thus to simulate what occurs in vivo. In the case of cumene hydroperoxide (oxidizing agent), a constant concentration of 100 μM was selected for all experiments.
3.2. Pyridoxine Decreases Methemoglobin Levels and Lipid Peroxidation in Erythrocytes
The concentration of metHb and MDA was lower for samples pretreated with pyridoxine compared to those not treated with pyridoxine (Table 2). Cumene hydroperoxide at 100 μM significantly increased lipid peroxidation (MDA= 10.14 ± 0.83 µM) in erythrocytes compared to the control without any treatment (MDA= 4.44 ± 1 µM). Pyridoxine at concentrations of 1, 10 and 100 μM significantly decreased lipid peroxidation of erythrocytes compared to the samples not treated with pyridoxine and subjected to oxidative stress with cumene hydroperoxide. The percentages of MDA-based oxidation inhibition were found to be 19, 21 and 24% respectively for each concentration of pyridoxine. No significant difference was found between the samples without any treatment and the samples only treated with pyridoxine, both for the levels of MDA and those of lipid peroxidation (p> 0.05, Student's t-test).
% Hemolysis | ||||
---|---|---|---|---|
Concentration (µM) | 2 h | 10 h | 24 h | |
HCum | 0 | 0.55 ± 0.012 | 2.34 ± 0.02 | 2.16 ± 0.12 |
0.1 | 0.30 ± 0.031 | 2.21 ± 0.12 | 2.04 ± 0.11 | |
1 | 0.59 ± 0.50 | 2.18 ± 0.05 | 1.92 ± 0.06 | |
10 | 0.46 ± 0.16 | 2.13 ± 0.05 | 2.07 ± 0.05 | |
100 | 0.68 ± 0.074 | 2.47 ± 0.21 | 2.31 ± 0.39 | |
1000 | 4.21 ± 1.02 | 20.2 ± 1.24 | 41 ± 3.15 | |
Pyridoxine | 0 | 0.85 ± 0.20 | 2.73 ± 0.40 | 2.63 ± 0.81 |
0.1 | 0.51 ± 0.15 | 2.71 ± 0.45 | 2.06 ± 0.23 | |
1 | 1.37 ± 1.12 | 2.73 ± 0.53 | 2.26 ± 0.14 | |
10 | 0.63 ± 0.21 | 2.49 ± 0.34 | 2.42 ± 0.34 | |
100 | 0.68 ± 0.25 | 2.98 ± 0.75 | 2.43 ± 0.09 | |
1000 | 0.53 ± 0.09 | 2.75 ± 1.62 | 1.89 ± 0.14 |
Samples | Pyridoxine (µM) | Hcum (µM) | % metHb | MDA (µM) |
---|---|---|---|---|
Control † | 0 | 0 | 7.22 ± 0.31 | 4.44 ± 1.00 |
Hcum ¶ | 0 | 100 | 9.32 ± 0.08 | 10.14 ± 0.83 |
Px1 ** | 1 | 100 | 8.15 ± 0.08 | 8.18 ± 0.91 |
Px 10 ** | 10 | 100 | 8.19 ± 0.05 | 7.96 ± 0.16 |
Px 100 ** | 100 | 100 | 7.44 ± 0.15 | 7.69 ± 0.83 |
Control Px1 | 1 | 0 | 7.09 ± 0.17 | 3.94 ± 0.80 |
Control Px 10 | 10 | 0 | 7.01 ± 0.23 | 4.01 ± 0.73 |
Control Px 100 | 100 | 0 | 7.26 ± 0.01 | 4.24 ± 0.69 |
3.3. Pyridoxine Decreases the Carbonylation of Proteins in the Erythrocyte Membranes
Cumene hydroperoxide at 100 μM significantly increased the carbonylation of membrane proteins (carbonylated proteins = 26.7 ± 6.39 nmol/mg) in erythrocytes compared to the control without any treatment (1.98 ± 0.88 nmol/mg). Pyridoxine at concentrations of 1, 10 and 100 μM significantly decreased the carbonylation of erythrocyte membrane proteins in comparison to the erythrocytes not treated with pyridoxine and subjected to oxidative stress with cumene hydroperoxide (Fig. 1). Control samples containing only pyridoxine showed similar behavior to the control without any treatment (P > 0.05, Student's t-test). The percentages of oxidation inhibition were found to be 57, 66 and 74% respectively for each concentration of pyridoxine.
3.4. Electrophoretic Analysis and Western Blot of Carbonylated Proteins
All samples incubated with cumene hydroperoxide had a different electrophoretic profile than the control sample. It was observed that for these samples, there was a notable possible presence of agglomerates of modified globin chains despite the previous process of washing and extracting membrane proteins (Fig. 2a). Cumene hydroperoxide is a very strong oxidant and causes molecular derangements in the hemoglobin chains. In the western blot analysis, a decrease in the oxidation signal intensity for the samples pretreated with pyridoxine was again demonstrated and additionally it was observed that oxidation by carbonylation of proteins was presented specifically for proteins with molecular weight higher than hemoglobin (Fig. 2b).
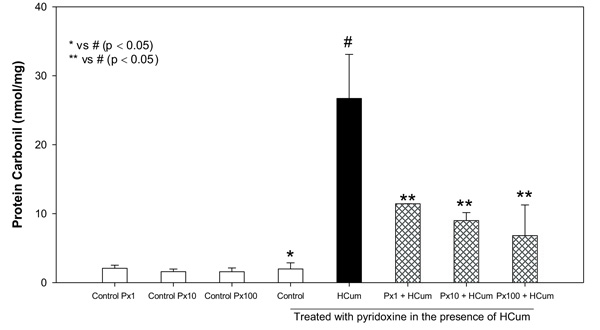
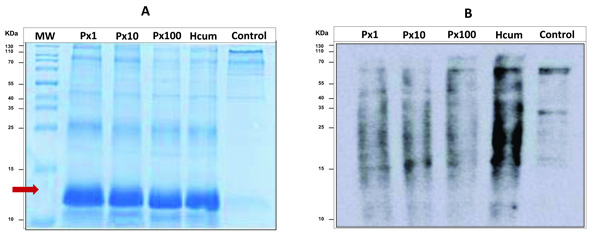
4. DISCUSSION
In both in vitro and in vivo studies, evidence of an additional role of pyridoxine has been obtained, acting in favor of the cellular antioxidant system, decreasing the levels of oxidative stress induced by different factors, especially inhibiting the production of superoxide radicals [5, 10] singlet oxygen [11] and lipid peroxidation levels [10, 40]. However, the mechanism is still not well understood.
Additionally, as a result of a theoretical study, it was reported that pyridoxine has a high probability of reaction with the radical HO•. In this computational theoretical model, it was obtained that the most favorable reaction was the subtraction reaction of an •H by this radical in the CH2OH groups or the OH group of the pyridine ring [7]. The superoxide anion showed little probability of experiencing any of the reactions evaluated with pyridoxine [7].
In the present study, the efficacy of pyridoxine was investigated against the oxidative stress induced by cumene hydroperoxide in human erythrocytes. Treatment with cumene hydroperoxide caused an increase in the levels of methaemoglobin, lipid peroxidation and protein carbonylation in erythrocytes treated only with this compound. However, these values were significantly lower in erythrocytes pretreated with pyridoxine before the addition of the oxidizing agent.
Methaemoglobin is synthesized at relatively low levels during the normal process of oxygen transport [1]. The exposure of the erythrocytes to cumene hydroperoxide allowed the oxidation of hemoglobin at higher than normal levels, producing substantial levels of methaemoglobin even for samples pretreated with pyridoxine, which was visually remarkable for the brown color that characterizes the presence of this oxidized form of hemoglobin. Even so, these levels were significantly lower compared to the levels of methemoglobin in erythrocytes exposed only to cumene hydroperoxide.
In general, the potency of a compound to act as an antioxidant is determined by several factors, such as the intrinsic chemical reactivity of the antioxidant towards the reactive species. The presence of functional groups such as -OH and -NH2 has been proposed to be responsible for the uptake and removal of oxygen radicals [41]. Both groups are present as substituents in the pyridine ring of pyridoxine, but further studies are needed to affirm that these two groups are responsible for the protective effect of oxidative damage manifested by pyridoxine.
Currently, there is no specific study that directly relates to the presence of pyridoxine and the reduction of the level of oxidation of erythrocyte membrane proteins and directed to protein carbonylation. The oxidation of proteins causes a modification in their structure and function. These oxidations are the mechanism by which oxidative stress has been related to the loss of physiological functions that occur under aging and certain chronic diseases [42]. The free radicals involved in the oxidation of proteins can act on their main structure or on the amino acid residues of their side chains (Fig. 3). The HO• radical has been shown to be a good mediator of oxidative protein modification [43]. In this way, the effect of pyridoxine on the oxidation of proteins obtained in this research could be explained by uptake of these radicals by this vitamin (Fig. 3).
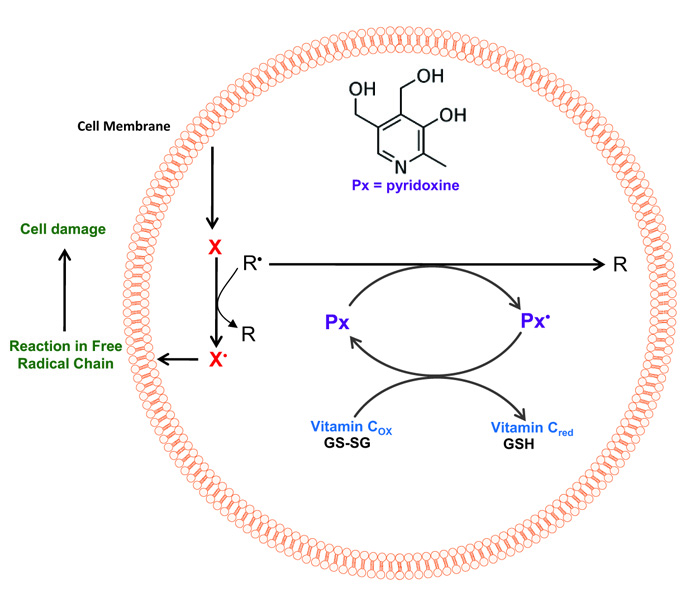
Finally, the results indicate that the presence of pyridoxine increases antioxidant protection in erythrocytes, by decreasing the levels of methaemoglobin, lipid peroxidation and carbonylation of proteins in erythrocyte membranes exposed to high concentrations of an oxidizing agent. This protective system is of relevance in the human organism; human cells lack the enzymatic system to perform the de novo synthesis of pyridoxine, therefore it depends exclusively on the vitamin coming from the diet. This could explain the susceptibility to oxidative stress of the human organism in comparison with other species.
CONCLUSION
The results obtained showed a significant reduction of oxidative stress markers, in the presence of pyridoxine. Until now, this effect has not yet been reported in terms of carbonylation of membrane proteins of human erythrocytes, which is the basis for suggesting which pyridoxine exerts a protective effect against oxidative damage in human erythrocytes.
ETHICS APPROVAL AND CONSENT TO PARTICIPATE
Ethical Approval was given by the Ethics committee of the University of Cartagena (Colombia).
HUMAN AND ANIMAL RIGHTS
No animals were used in this research. All human research procedures followed were in accordance with the ethical standards of the committee responsible for human experimentation (institutional and national), and with the Helsinki Declaration of 1975, as revised in 2013.
CONSENT FOR PUBLICATION
Not applicable.
AVAILABILITY OF DATA AND MATERIALS
The authors confirm that the data supporting the findings of this research are available within the article.
FUNDING
This study was financially supported by the Vice-Rectory of Research of the University of Cartagena, Cartagena, Colombia through the research grant (1929-2011 and 003334-2018) to conduct this work.
CONFLICT OF INTEREST
The authors declare no conflict of interest, financial or otherwise.
ACKNOWLEDGEMENTS
The authors would like to thank the research management center at University of Cartagena for providing us with the finical support.