All published articles of this journal are available on ScienceDirect.
Antiproliferative Effects of Ellagic Acid on DU145 Cells
Abstract
Background:
Prostate Cancer (PC) represents a leading cause of tumor-related death among men in the Western world. Above all, DU145 cell line represents the most particular cells model of PC, derived from a central nervous system metastasis. In recent years, functional and healthy diet has gained a pivotal role in society, allowing the possibility to deal with cancer before its emergence or progression, profiting by anti-tumor properties of dietary phytochemicals. Among them, Ellagic Acid (EA) is found in several fruits and vegetables, whose juice demonstrated antioxidant, anti-carcinogenic and anti-fibrotic properties.
Methods:
DU145 prostate cancer cell line was used to determine the effects of ellagic acid on cell viability. In order to evaluate metastatic feature of DU145, VEGF-A and OPG levels by ELISA assay were assessed. Expression of β-catenin, HO-1, HO-2 and SIRT1, markers of proliferative and defense capacities, were determined by western blotting. To strengthen the study, cell transfection with siRNA β-catenin was performed.
Results:
In the presence of EA, the viability of DU145 cells was reduced by about 40 and 50%, respectively after the exposure to 50 and 100 μM concentrations. We also observed a reduction of both levels of VEGF-A and OPG, confirming the important role of EA in facing the metastasis development. EA treatment (50 μM) induced a significant reduction of β-catenin and SIRT1 levels and, similarly, there was a decrease of HO protein expression, more pronounced for HO-2, showing EA activity on the proliferative feature of DU145 cells. Knockdown of β-catenin by siRNA, in the presence of EA treatment, inhibited cell proliferation.
Conclusion:
Ellagic acid exhibits significant antiproliferative effects in our in vitro model of prostate cancer’s metastasis, suggesting that, the use of EA as a multitarget natural compound, may represent a possible strategy for cancer chemoprevention.
1. INTRODUCTION
Prostate Cancer (PC) is one of the most common cancer among men and represents a leading cause of tumor-related death, especially in the western world [1-3]. Because of the similarity between PC’s and benign prostate disease’s early symptoms, several patients are treated only in the advanced stage of the tumor progression [4]. Exceeding this point, therapy resistance usually occurs in PC, generating distant metastasis [5]. Patients with high-risk prostate cancer, even in case of radical prostatectomy, present critical conditions due to the presence of delocalized forms of the tumor [6]. During the first steps, genetic alterations and a supporting tumor microenvironment are necessary to confer metastatic status to prostatic cells, permitting displacement toward surrounding tissues [7]. Among all organs compromised by PC’s metastasis, the osteogenic niche makes the bone the most important site of cancer cell proliferation [8, 9]. In fact, a complex cytokine system (RANKL, RANK, OPG) is involved in bone’s resorption and turnover: this seems to be the key point that explains the favorable microenvironment for PC’s cells growing [10]. There are few cell lines available to analyze the metastatic feature of PC and, above all, DU145 cell line represents the most particular cells model that is derived from a central nervous system metastasis, although finding its origin in prostate carcinoma [11, 12]. For this reason, DU145 cells can be chosen to explain the peculiar capacity of PC cells to be invasive and easily capable to move from starting location [13]. The development of PC is a process due to many factors involved in cell's growth and division, thus, inducing a delay or an inhibition of these processes, especially exploiting natural substances commonly present in the diet, could be the right way to prevent cancers from turning clinically significant [14-16]. In recent years, the increasing role of functional and healthy diet allows the possibility to reduce the application of specific drugs that, as cytotoxic agents, are associated to many side-effects that may worsen the quality of patient life [17, 18]. The attention for chemoprevention, thanks to new human acceptance, permits to deal with face cancer before its emergence or progression profiting by anti-tumor properties of dietary phytochemicals, eventually used in combination with treatments of choice [19-21]. Several natural compounds, in particular polyphenols, exhibit an antioxidant activity both in vitro and in vivo [22]. Among them, Ellagic Acid (EA) is found in several fruits and vegetables, like strawberries, blackberries, nuts, and especially in the pomegranates, whose juice demonstrated antioxidant, anti-carcinogenic and anti-fibrotic properties [23-25]. For these reasons, EA has been tested against several cancer types: studied for breast cancer therapy, it inhibited the proliferation and migration of SUM159 and HCC1954 breast cancer cells [26]; as a consequence of p53 activation, it induced growth reduction in colon cancer SW480 cells because of DNA damage [27]; it was also used for bladder cancer, to which it showed an inhibition of extracellular matrix invasion of human bladder cancer cells in response to VEGF-A [28]. The latter, the Vascular Endothelial Growth Factor - A, is the first mediator of normal and pathological angiogenesis, associated with disease progression and recurrence [29]. High levels of VEGF-A are expressed by many tumors, including PC, in which the growth possibility is extended even in metastatic sites.
The aim of this study is to demonstrate the effect of EA treatment on prostate cancer DU145 cell viability and on some specific markers involved in metastatic invasion and migration.
2. MATERIALS AND METHODS
2.1. Cell Culture
DU145 cells (Human prostate carcinoma, epithelial-like cell line) were purchased from American Type Culture Collection (Manassas, VA, USA) and grown in DMEM supplemented with 10% Fetal Bovine Serum (FBS), 0,1% streptomycin-penicillin, 1% L-glutamine and 1% non-essential amino acids. Cells were incubated at 37° C in a 5% CO2 humidified atmosphere and maintained at sub-confluency by passaging with trypsin-EDTA (Gibco, NY, USA).
2.2. Cell Viability Assay
DU145 cells were seeded at a concentration of 2×105 cells per well of a 96-well, flat-bottomed 200-μl microplate. Cells were incubated at 37°C in a 5% CO2 humidified atmosphere and cultured for 48h in the presence and absence of different concentrations (5–100 μM) of EA (≥95% - HPLC – powder, from tree bark) (Sigma-Aldrich. ST Louis. MO-USA). Four hours before the end of the treatment time, 20 μl of 0.5% 3-(4,5-dimethylthiazol-2-yl)-2,5-diphenyltetrazolium bromide (M TT) in Phosphate-Buffered Saline (PBS) was added to each microwell as previously described [30]. The optical density was measured using a microplate spectrophotometer reader (Thermo Labsystems Multiskan, Milano, Italy) at λ=570 nm.
2.3. VEGF-A and OPG Measurements
Cells were seeded at a constant density to obtain identical experimental conditions in the different tests and to achieve a high accuracy of the measurements. VEGF-A and OPG levels were determined in the culture supernatant using an ELISA kit (AssayGate, Ijamsville, MD, USA). The assays were performed according to manufacturer’s guidelines. Results were expressed as pg/mL.
2.4. Immunoblotting of Signaling Proteins
Cells were trypsinized, pelleted by centrifugation, and lysed with lysis buffer supplemented with protease and phosphatase inhibitors (complete™ Mini and PhosSTOP™; Roche Diagnostics, Indianapolis, IA). Protein levels were quantified using a commercial assay (Bio-Rad, Hercules, CA). Protein samples were applied to Sodium Dodecyl Sulfate (SDS) polyacrylamide gel (10%–15%), electrophoresed under denaturing conditions, and electrotransferred onto PVDF Immobilon-P membrane (Amersham Pharmacia, Piscataway, NJ) using a semidry transfer apparatus (Bio-Rad). Membranes were blocked with Odyssey® Blocking Buffer (TBS) (LI-COR, Lincoln, NE) for 1 h at room temperature. Primary antibodies (1:500 – 1:1,000 dilution) in blocking buffer supplemented with 0.1% Tween 20 (Fisher Scientific) were incubated overnight at 4°C, washed in Tris-Buffered Saline (TBS) supplemented with 0.1% Tween 20, and then incubated with the appropriate fluorophore-conjugated secondary antibodies (1:5000–1:20000) (LI-COR). Detection and quantification of signals were completed with a LI-COR Odyssey instrument (LI-COR).
2.5. siRNA Transfection
Transfection was carried out using a solution mix composed of transfection reagent and β-catenin siRNA diluted in siRNA transfection medium (Santa Cruz Biotechnology). DU145 cells were incubated with the solution mix for 5h and then DMEM supplemented with 20% FBS and 1% penicillin /streptomycin was added for the next 24h. After incubation, the cells were treated with EA (50μM) for 48h. A non-targeting siRNA solution (Ambion) was used as negative control.
3. RESULTS
3.1. Effect of Ellagic Acid on Cell Viability
The viability of DU145 cells, in the presence of EA, was assessed by the MTT assay, testing three different concentrations (5, 50, 100 μM). After 48h of EA treatment, the lowest examined concentration did not demonstrate a significant cytotoxic effect, comparing with the untreated cells representing the control group. Contrary, as seen in Fig. (1), we observed a noticeable loss of vitality after EA treatment at the highest concentrations. In particular, cell viability was reduced by about 40 and 50% after the exposure respectively to the 50 and 100μM.
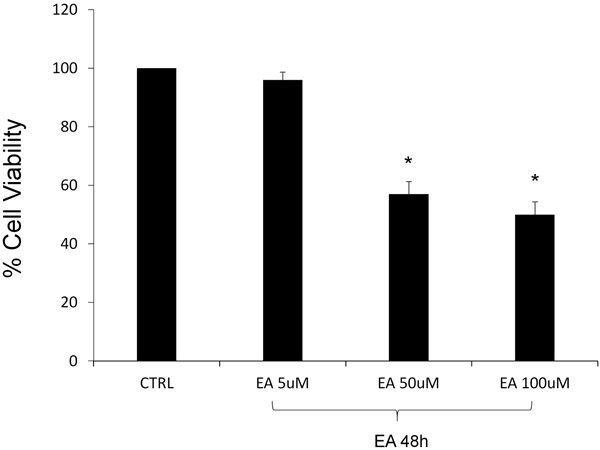
3.2. Effect of Ellagic Acid on VEGF-A and OPG
Prostate cancer is known to be associated with bone metastases. Additionally, the involvment of angiogenic factors related to cell proliferation is common. In order to consider these typical features, we measured the VEGF-A and OPG levels in conditioned media obtained after EA treatment (50 μM). The ELISA assay (Fig. 2) showed a decrease of both proteins, confirming the hypothesis about the effect of EA on the release of these factors.
3.3. Effect of Ellagic Acid on β-catenin, HO-1, HO-2 and SIRT1 Levels
To evaluate the suppression of proliferative and defence capacities of DU145 cells after EA treatment, we assessed the levels of β-catenin, HO-1, HO-2 and SIRT1 (Fig. 3). The densitometric analysis, after normalization with beta-actin, showed that EA treatment, at the concentration previously tested (50 μM), induced a significant reduction of β-catenin and SIRT1 levels. Similarly, we observed a decrease of Heme Oxygenase (HO) protein expression, particularly marked for the constitutive isoform (HO-2).
3.4. Effect of Silencing β-catenin on Cell Proliferation
The effect of EA on cell proliferation was tested after silencing β-catenin gene. DU145 cells were treated with EA (50 μM) for 48h in the presence and absence of siRNA against β-catenin; cell viability was detected by MTT assay. Fig. (4) shows that β-catenin siRNA caused a slight decrease in cell viability, while scrambled siRNA did not demonstrate any toxicity. The combination of EA and β-catenin siRNA induced a significant additional reduction of cell viability compared to EA group.
4. DISCUSSION
Prostate cancer is a solid tumor that owns some peculiar features: it is difficult to diagnose in early stages, it shows very high resistance during treatment with chemotherapeutical drugs and, because of its inclination to develop metastasis, the choice of the best drug is complicated and influenced by properties of new affected tissues. This condition leads to patients to embrace new strategies that, in last years, concern chemoprevention and using bioactive compounds. Among natural polyphenols we tested on DU145 PC cells, EA was the best capable compound to face PC’s progression, acting on some specific markers. In the early phase, we assessed EA’s activity on DU145 cells viability, evaluating mitochondrial damage in these cells by MTT assay. Obtained results confirmed our previous study [31], showing a halving of cells viability compared to untreated control, using the highest concentration of EA (50-100 μM). Fig. (1) shows the functional activity of EA stopping cells growth, inhibiting their proliferation. The possible action on mitochondrial function was reported by a similar study, based on EA’s effect on colon cancer Caco-2 cells, that demonstrated a comparable loss of viability on these cells [32]. Although tested in different cell lines and indepen dently of chosen concentrations, EA leads to a halt on cancer cells proliferation, demonstrating the central role of mitochondria in cancer cells survival [33]. Known the EA’s adapt concentration to observe an efficacy on DU145 cells, our analysis continued evaluating resistance and migration features, proper of PC cells [34]. β-catenin is considered part of a protein complex involved in signal transduction that regulates tissues development and biological processes [35, 36]. This pathway is mediated by the Wingless-type (Wnt) proteins, and influences cell apoptosis and tumorigenesis [37, 38]. Wnt protein, binding its cell surface receptor Fz (Frizzled), triggers a signalling cascade through β-catenin, that induces CyclinD1 gene, involved in cell proliferation [39-41]. Is also known the association between β-catenin and the cell adhesion molecule E-cadherin, that represents an essential factor during migration phase of cancer progression [42]. A recent study reported the efficacy of quercitin, another natural compound, on β-catenin signal, whose reduction is associated with the arrest of carcinogenic process, leading to an antiproliferative and apoptotic effect on colon cancer cell lines [43, 44]. Similarly, our results demonstrate a strong reduction of β-catenin protein expression, suggesting that EA could reduce DU145 cells proliferation inhibiting cancer progression. We next investigated whether silencing β-catenin could affect the reduction of cell proliferation mediated by EA. As shown in Fig. (4), and according to previously published results [45-47] pre-treatment with siRNA β-catenin enhanced anti-proliferative effect of EA. SIRT-1 is a member of the sirtuin family of histone deacetylase that is nicotinamide adenine dinucleotide (NAD+)-dependent, and is involved in aging and in cancer growth [48, 49]. The role of SIRT1 as oncogenic protein is explained by the list of its substrates, inhibited by deacetylation, whose function as tumor suppressors is inactivated: Bax, Ku70, FOXO, the Retinoblastoma (Rb) protein, and p53 [50-52]. It has been reported that SIRT1 is significantly overexpressed in many types of cancer, including colon cancer, acute myeloid leukemia and prostate cancer [53-55]. Our results suggest that EA could play a significant role in the reduction of DU145 cells growth and viability even through SIRT1 inhibition, permitting to onco-suppressor genes to induce apoptosis in cancer cells. Many cancers, including PC, overexpress their cytoprotection system leading to stronger resistance to physiological defence or drugs effects. Several studies reported that Heme Oxygenase (HO) system seems to play a major role in cancer cell protection, guaranteeing regulation for redox homeostasis [56-60]. Normal and tumor cells own two different isoforms of HO: one of them (HO-1), is inducible by oxidative stress or inflammatory conditions [61]; the other one (HO-2) represents the constitutive isoform, contributing to basal physiological functions including a decrease in oxidative stress, inflammation, and protection against apoptosis. The main role of HO is to catalyse the rate-limiting step in heme degradation, causing the production of Carbon Monoxide (CO), iron and biliverdin; besides that HO system has been also involved in the development of drug-resistance in various tumors [62-65]. After EA treatment, we observed a strong reduction of HO levels which it is more pronounced for constitutive isoform, showing high capacities of EA to decrease the basal defence features of PC cells, in which progression is known HO involvedness [66]. Inhibiting overexpression of both inducible and constitutive isoforms of HO and the previously mentioned SIRT1, EA demonstrated its contribution to decrease cytoprotective system in DU145 cells, perhaps activating apoptotic pathways that could stop PC cells growth [67]. Every type of cancer cells, including PC cells, needs to develop new vascular structures in surrounding tissues, to guarantee their own survival. This serious condition shows an invasive step of cancer growth, especially associated with metastasis, that permits cancer cells to become part of new tissue, fitting in different location. VEGF-A is the most important factor involved in cancer angiogenesis, stimulating endothelial cells proliferation and formation of new capillary vessels tumor-related [68-71]. In Fig. (2), we observed a statistically significant decrease of VEGF-A level in presence of EA, confirming our previous results obtained on PC LnCap cells [72]. The efficacy of EA on DU145 cells was also demonstrated evaluating the release of Osteoprotegerin (OPG), a peculiar marker involved in bone metastasis. In healthy bone, osteoblastic cells play a central role in bone turnover through the regulation of osteoclastogenic activity [73]. This microenvironment is modified in the presence of PC cells, producing specific effectors that allow an unchecked growth [74]. One of them, OPG, inhibits osteoclastogenesis, avoiding bone remodeling and permitting bone cells to proliferate [75, 76]. It has been reported that PC cells release specific exosomes, which content is transferred to target cells [77]. Different miRNAs, contained in these vesicles, seem to promote tumor metastasis [78] and, one in particular (miR-141-3p), is transferred to osteoblasts promoting their activity, leading to the formation of a bone-metastasis microenvironment. Interestingly, results show an increased OPG release by osteoblast activity, confirming their importance in tumor progression [79]. An altered balance between osteoblast and osteoclast promotes metastatic potential in human prostate cancer [80]. Reduction of OPG levels in DU145 cells culture medium after treatment with EA, demonstrates the role of EA in restoring bone microenvironment and exploiting osteoclastic cell activity to reduce tumor growth.
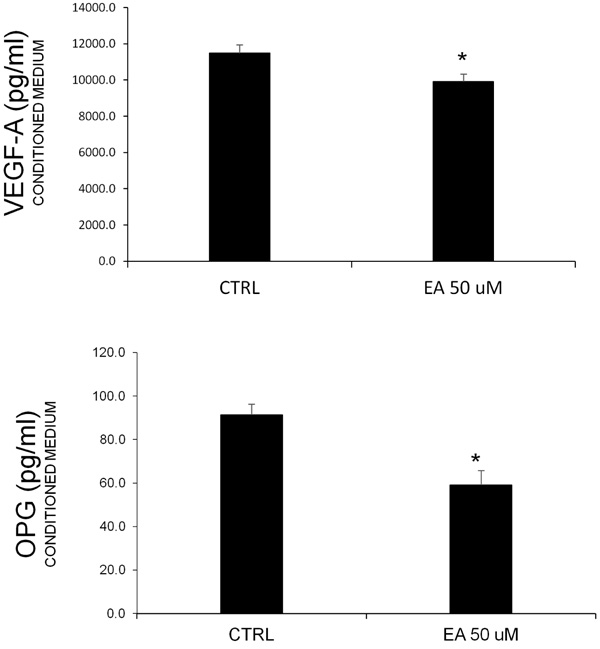
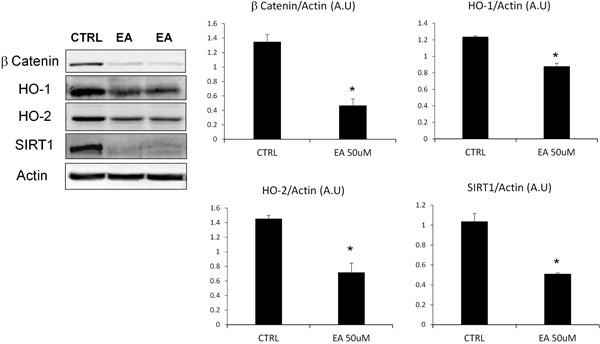
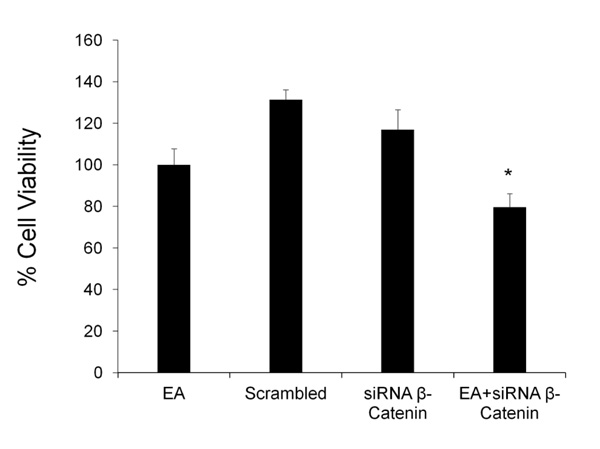
CONCLUSION
The present study shows the importance of ellagic acid, as a multivalent natural compound, inhibiting DU145 proliferation through different biochemical mechanisms. Our results suggest that EA could reduce main features of PC related to invasiveness, angiogenesis and metastatic dislocation, noticeable from modulation of factors involved in these processes. In conclusion, we demonstrated that EA treatment may represent an innovative strategy for cancer chemoprevention, supporting traditional therapies.
ETHICS APPROVAL AND CONSENT TO PARTICIPATE
Not applicable.
HUMAN AND ANIMAL RIGHTS
No animals/humans were used for studies that are the basis of this research.
CONSENT FOR PUBLICATION
Not applicable.
AVAILABILITY OF DATA AND MATERIALS
Not applicable.
FUNDING
None.
CONFLICT OF INTEREST
The authors declare no conflict of interest, financial or otherwise.
ACKNOWLEDGEMENTS
Declared none.